Why do batteries age?
GC-MS analysis of fluorophosphates as possible early-stage quality indicators for batteries
Dr. Waldemar Weber, Shimadzu Europa GmbH
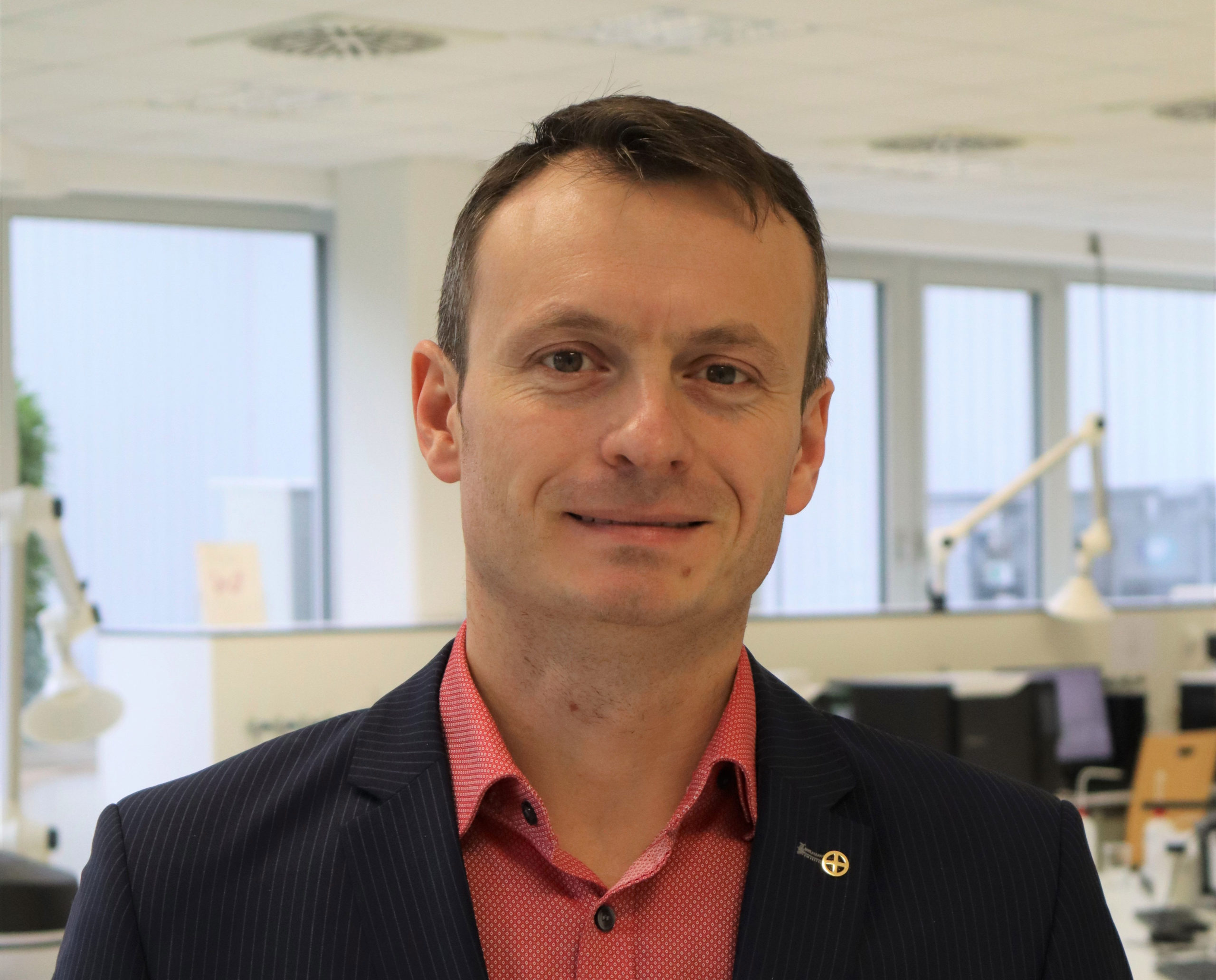
Especially fluor-containing organophosphates are of interest due to their potential neurotoxicity [4, 5, 6] While the electrochemical aging takes place in the battery itself, the chemical aging starts already during manufacturing, storage and transport of the electrolyte. Typical factors that influence this are: exposure to air and moisture, too high temperature during manufacturing/storage, long-term storage in general. Wrong materials in the production pipeline like glass are problematic as well due to a catalytic reaction of the fluoric acid traces with SiO2 as follows: SiO2 + 6HF → H2[SiF6] + 2H2O. This application is an example for the analysis of an electrolyte solution for the qualitative determination of fluorophosphates as a possibility for an early-stage quality control.
Sample preparation and measurement
25 µl LIB electrolyte consisting of ethyl methyl carbonate and ethylene carbonate (1:1) with 1M LiPF6 has been diluted with 1 mL dichloromethane and centrifuged for 5 min at 8,500 rpm to remove the solid LiPF6. The centrifuged solution was transferred into a 2-mL GC glass vial and stored at 7 °C until it was measured by a GCMS-QP2020 NX (Figure 2).
Method details
The analytical GC-MS conditions are listed in detail in Table 1.
System configuration | ||
---|---|---|
Model | GCMS-QP2020 NX | |
Autosampler | AOC-30i | |
GC Parameter | Injection mode | Splitless |
Column | SH-I-5MS (30 m x 0.25 mm x 0.25 µm; P/N 221-75940-30) | |
Lin. velocity | 36.8 cm/s | |
GC oven method | 35 °C for 1 min, 3 °C/min to 60 °C, 30 °C/min to 210 °C, 210 °C for 1 min | |
Transfer line temperature | 210 °C | |
Carrier gas | He | |
MS parameter | Ion source temperature | 180 °C |
Ionization mode | EI | |
Measurement mode | SIM/Scan | |
Solvent cut time | 2 min |
Decomposition mechanism of LIB electrolyte
The electrolyte used for this experiment was stored for around one year at -30 °C in a refrigerator, any aging processes under such conditions should be extremely slowed down. Nevertheless, such a long storage already has an influence on decomposition, as it is shown in Figure 4, a chemical rearrangement of ethyl methyl carbonate (EMC, 2) to dimethyl carbonate (DMC, 1) and diethyl carbonate (DEC, 3) could be observed. The decomposition of LiPF6 salt under reaction with traces of water and the corresponding electrolyte solvent can be influenced by electrochemical and chemical processes. The reaction mechanisms are basically identical, the content of the produced organophosphates is strongly dependent on the aging stage of the electrolyte/LIB.[2, 3] For the dissolved LiPF6 salt, a thermodynamic equilibrium LiPF6 ↔ LiF + PF5 is considered as a starting point for its destruction, where the PF5 is forming POF3 under reaction with traces of water followed by a chain of reactions of POF3 with organic carbonate solvents. The exact reaction mechanisms are still a point of intensive research. One of the proposed mechanisms from POF3 to fluorophosphates is shown in Figure 3. Summarizing, the destruction process can be divided in four steps, 1: creation of POF3, 2: creation of difluorophosphate, 3: creation of monofluorophosphates, 4: creation of trialkyl phosphates.
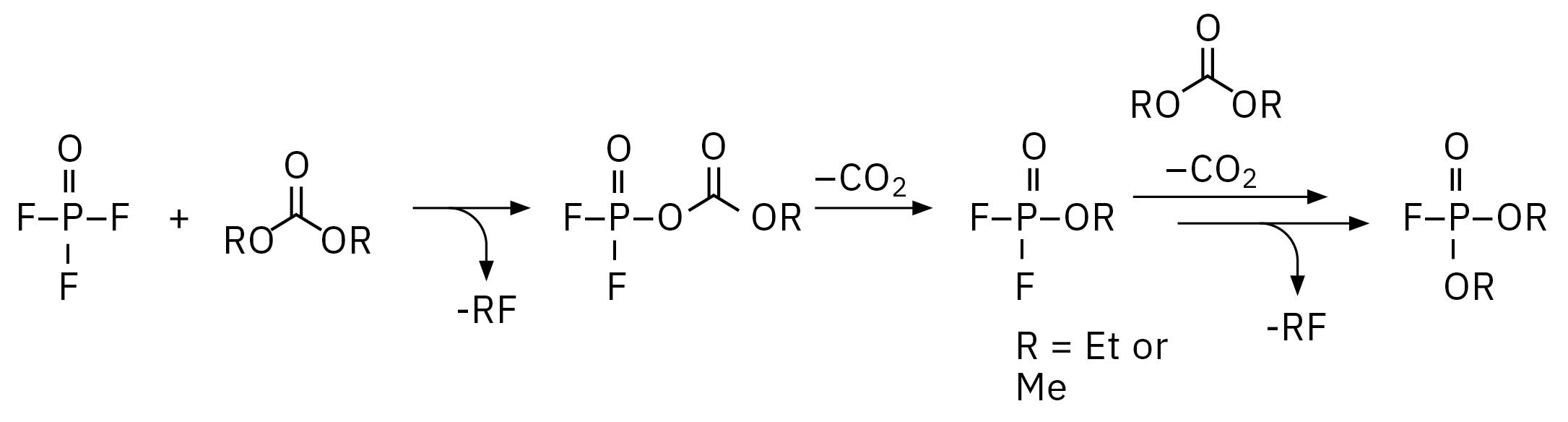
A simple and reliable method to identify early signs of destruction using liquid injection for GC-MS
To analyze the highly volatile destruction products of step 1 and 2, a headspace-based injection for GC-MS is required due to a coalition with the solvent. The liquid-based injection used for this application allows the analysis of the products in step 3 and 4. The used electrolyte consists of ethyl methyl carbonate, therefore methylated, ethylated and mixed ethyl methyl phosphate species can be expected. A SCAN/SIM mode for detection allows a clear identification of compounds by a mass spectrum and a sensitive and selective detection using SIM traces. The applied m/z values for SIM detection are collected in Table 2. For the analyzed electrolyte, only dialkylated species could be detected (Table 2) but no trialkylated ones. The reason is a decomposition at the very early stage as just long-term stored electrolyte was used, where the creation of trialkylated species has not started yet. The obtained mass spectrum is shown in Figure 5 and the corresponding chromatograms in Figure 6. The general problem for identifying such compounds is the absence of M+ peak in the spectra (except DMFP) and their absence in typical GC-MS libraries (except DEFP). To identify the spectrum, researchers used synthesized standards and for more complicated species NCI with CI GC-MS detections in addition.[3]
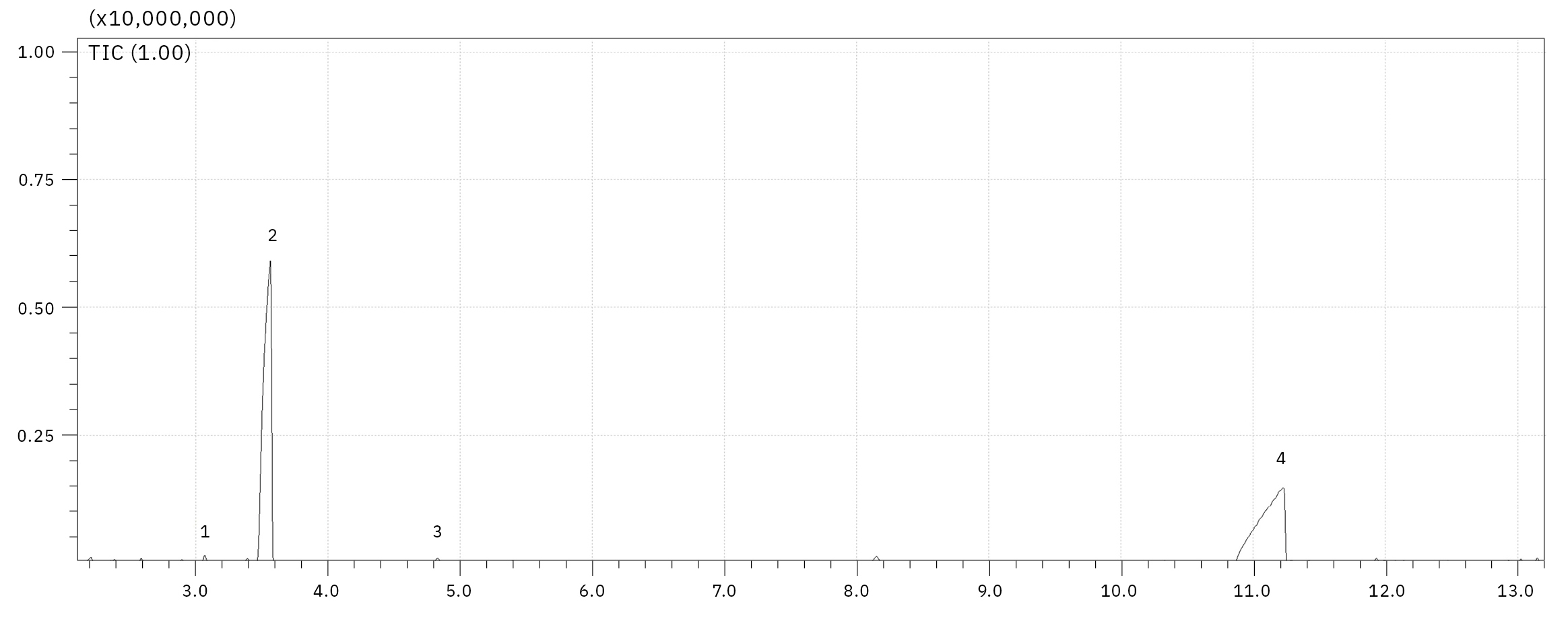
Compound |
Chemical structure |
m/z for SIM |
Ret. time [min] |
Dimethyl fluorophosphate (DMFP) |
97, 98, 128 |
4.44 |
|
Ethyl methyl fluorophosphate (EMFP) |
97, 115, 127, 141 |
6.3 |
|
Diethyl fluorophosphate (DEFP) |
101, 113, 129 |
9.0 |
Aiming for a prolonged battery life
LiPF6-based LIB electrolyte degrades during chemical and electrochemical processes. With the GC-MS-based analysis method a clear identification of three fluorinated alkyl phosphates has been successfully accomplished in a long-term stored electrolyte. It shows the suitability of the GCMS-QP2020 NX to offer a reliable and simple way of analysis even at early stages of electrolyte decomposition in order to make battery life more predictable and sustainable.
[1] X. Mönnighoff, Investigation of Lithium Ion Battery Electrolytes with Gas Chromatography after Recovery by Supercritical Fluid Extraction, PhD Thesis, University of Münster, 2020.
[2] Waldemar Weber, Ralf Wagner, Benjamin Streipert, Vadim Kraft, Martin Winter, Sascha Nowak, Journal of Power Sources, 2016, 306, 193–199.
[3] Waldemar Weber, Vadim Kraft, Martin Grützke, Ralf Wagner, Martin Winter, Sascha Nowak, 2015, 1394, 128–136.
[4] K. Husain, Delayed neurotoxicity of organophosphorus compounds, J. Environ. Immunol. Toxicol. 1 (2013), 14–21.
[5] L.D. Karalliedde, P. Edwards, T.C. Marrs, Variables influencing the toxic response to organophosphates in humans, Food Chem. Toxicol. 41 (2003), 1–13.
[6] A. Ordentlich, D. Barak, C. Kronman, N. Ariel, Y. Segall, B. Velan, A. Shafferman, The architecture of human acetylcholinesterase active center probed by interactions with selected organophosphate inhibitors, J. Biol. Chem. 271 (1996), 11953–11962.