Hot on the heels of infrared radiation
A novel experiment for measuring thermal radiation
Dr. Benjamin Thomas, Shimadzu Europa GmbH
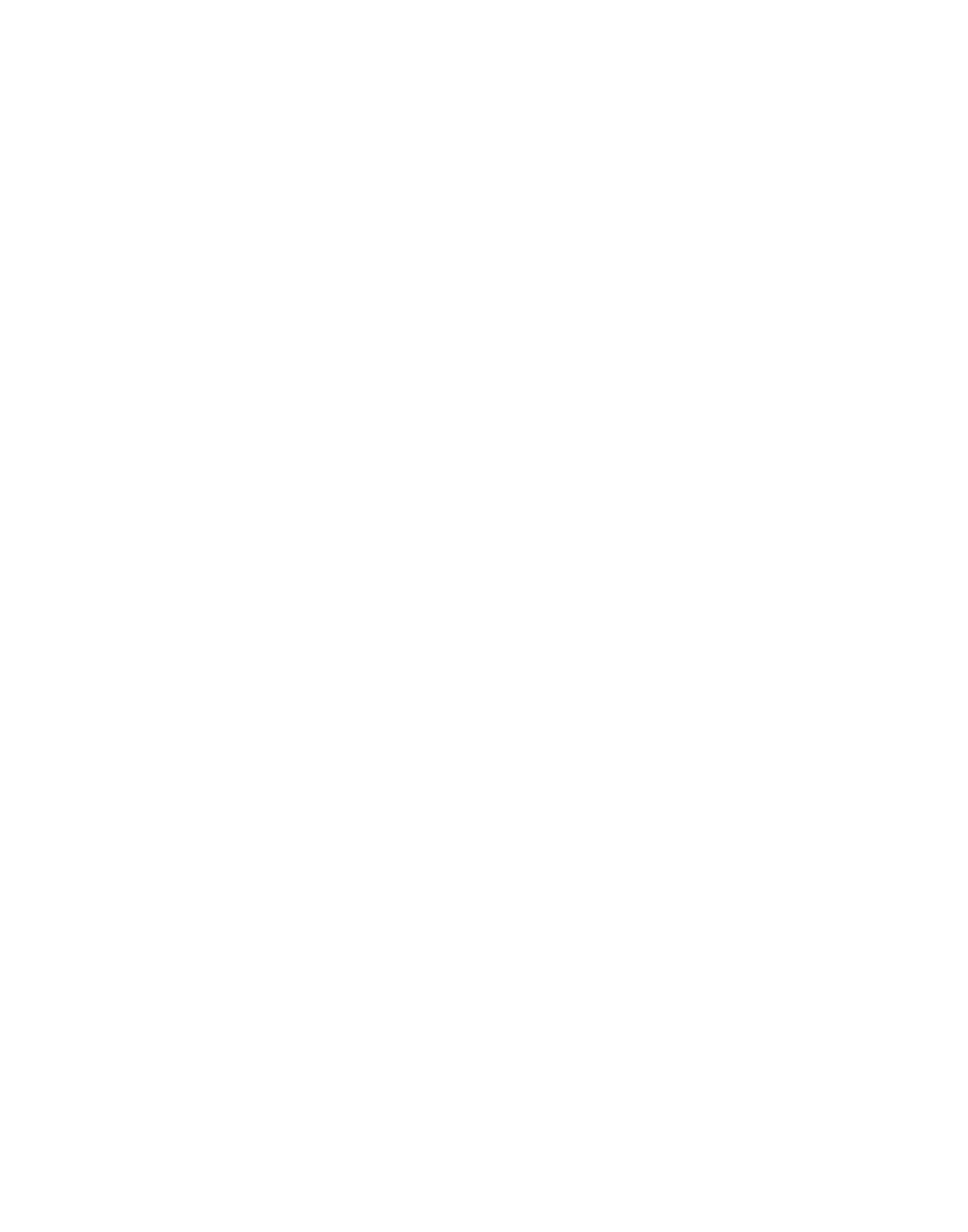
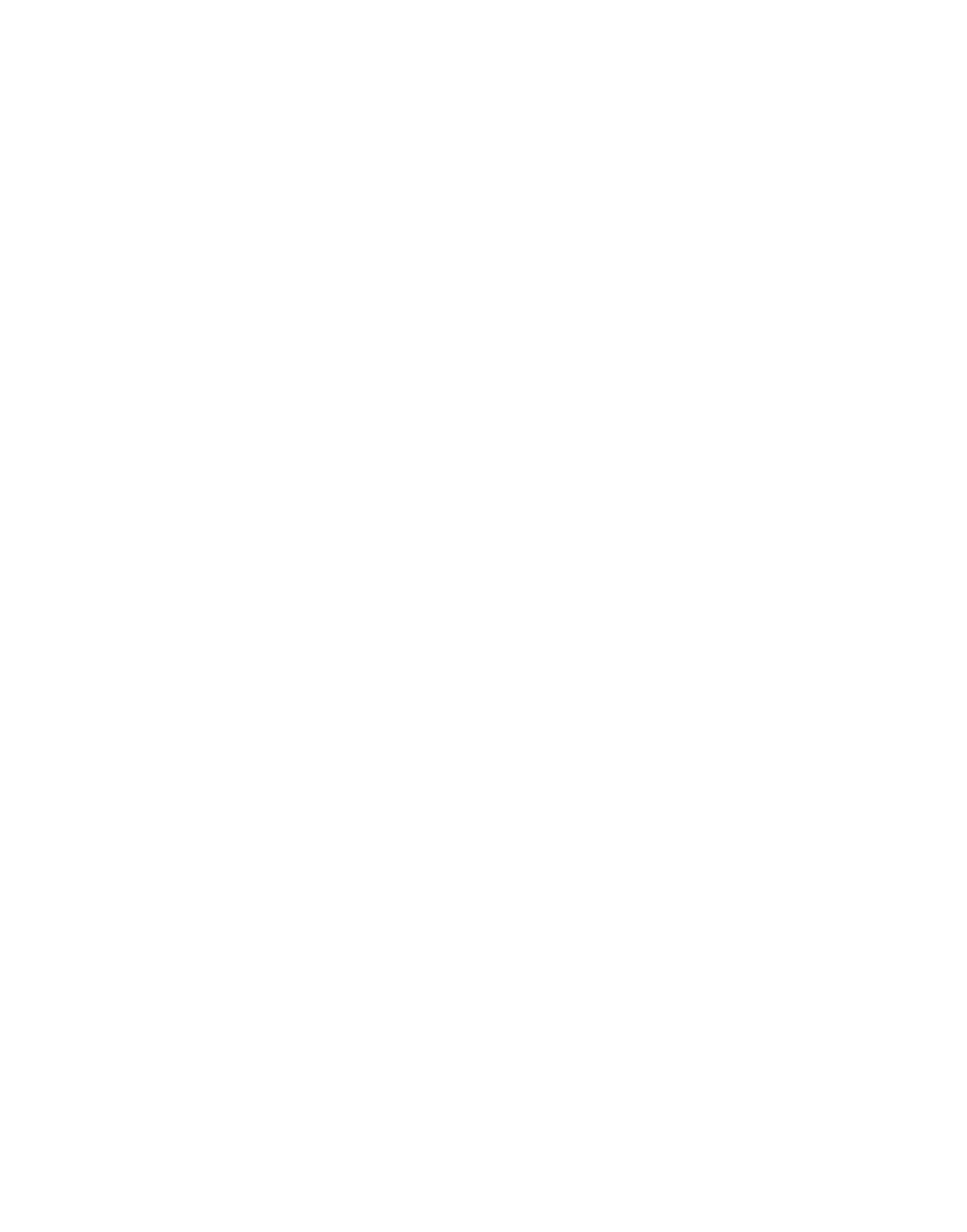
Heat transfer through infrared radiation occurs often in everyday life. Infrared heaters provide warmth in the living room, terrarium or canteen display. This type of heat transfer between particles also plays an important role in industrial processes, for example in iron processing or lime burning, where bulk material is heated in massive ovens.
One area of research at the department of Energy Plant Technology at Ruhr University Bochum, led by Martin Schiemann, is the investigation of this heat transfer between particles in packed beds. An arrangement of metal or mineral rods serves as a model system. A unique experiment based on a modified IRTracer-100 was developed for measuring the emissions.
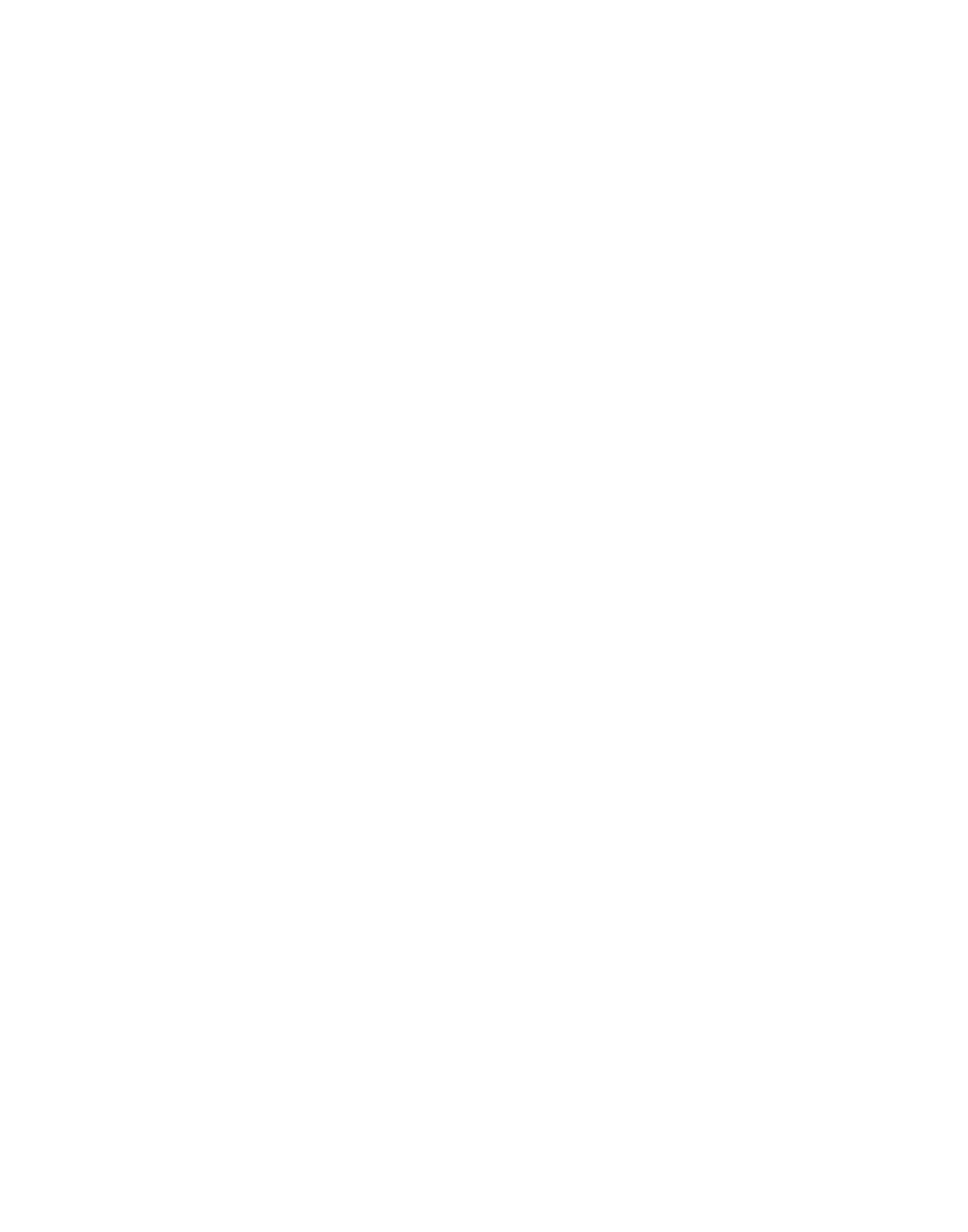
Heat transfer from a warmer body to a colder one can basically take place in three different ways, as shown (Figure 1) in the example of a pot of water:
(A) Via convection with mass transfer in a mobile medium
(B) Via thermal conduction through a medium without mass transfer
(C) Via thermal radiation, which is also possible in a vacuum
In convection (A), differences in temperature are followed by differences in density, which cause molecules to move from high-pressure zones (warmer) to low-pressure zones (colder). In this experiment, for example, hot water rises to the top of the pot. Temperatures in both layers equalize when the two kinds of water are mixed.
In thermal conduction (B), heat is transferred by vibrations (non-metallic solids), impacts (fluids) or mobile electrons (metals), depending on the nature of the medium. The “warmer” molecule transfers energy to the “colder” molecule here.
This article takes a closer look at thermal radiation (C), which is where a hot body emits broadband radiation in the infrared spectral range. When a material absorbs this infrared radiation, this stimulates vibrations and rotations there again, which in turn heats the absorbing material.
Thermal radiation is the only type of heat transfer that does not require a medium. However, the heat is only transferred if the material actually absorbs this infrared radiation and does not reflect it. For this reason, an evacuated chamber with mirrored surfaces is used for insulation, e.g. in thermos flasks, to prevent all types of heat transfer. Heat lamps, on the other hand, specifically generate infrared radiation to transfer heat.
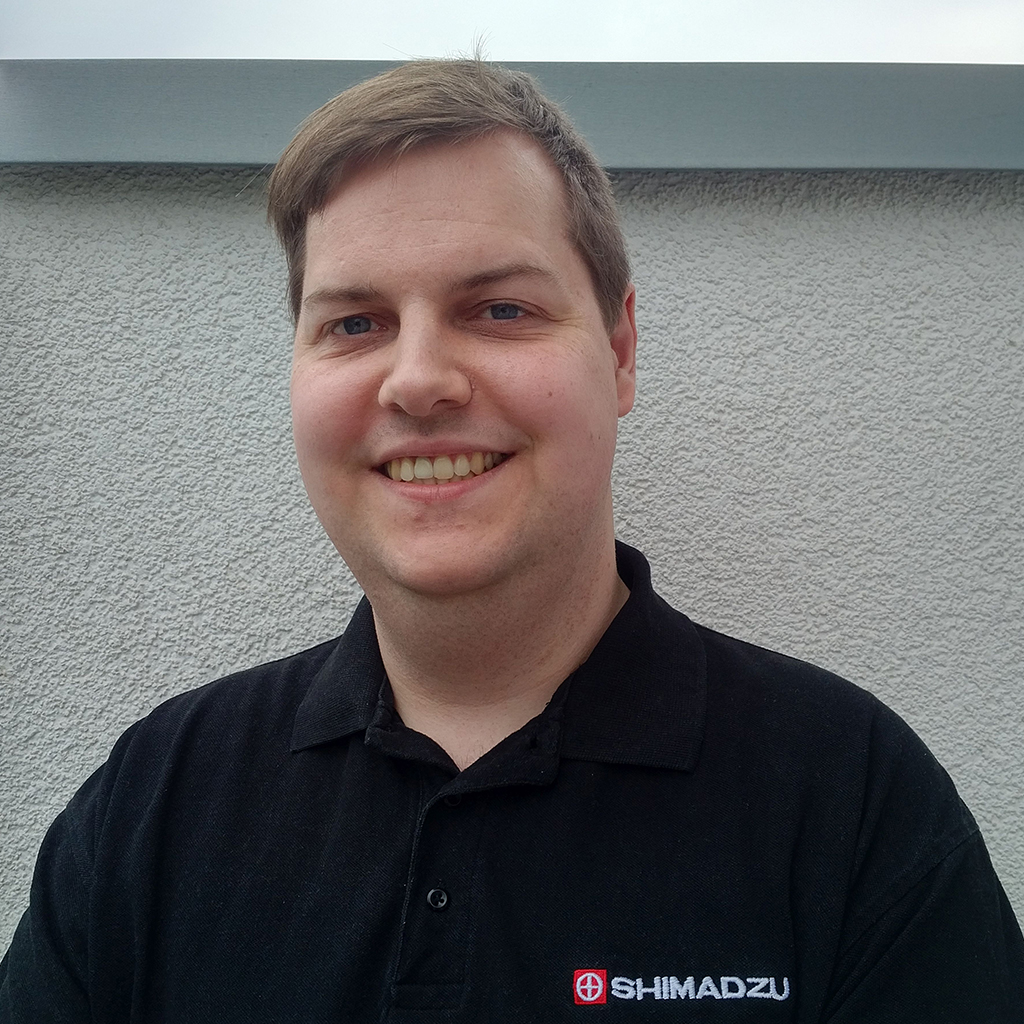
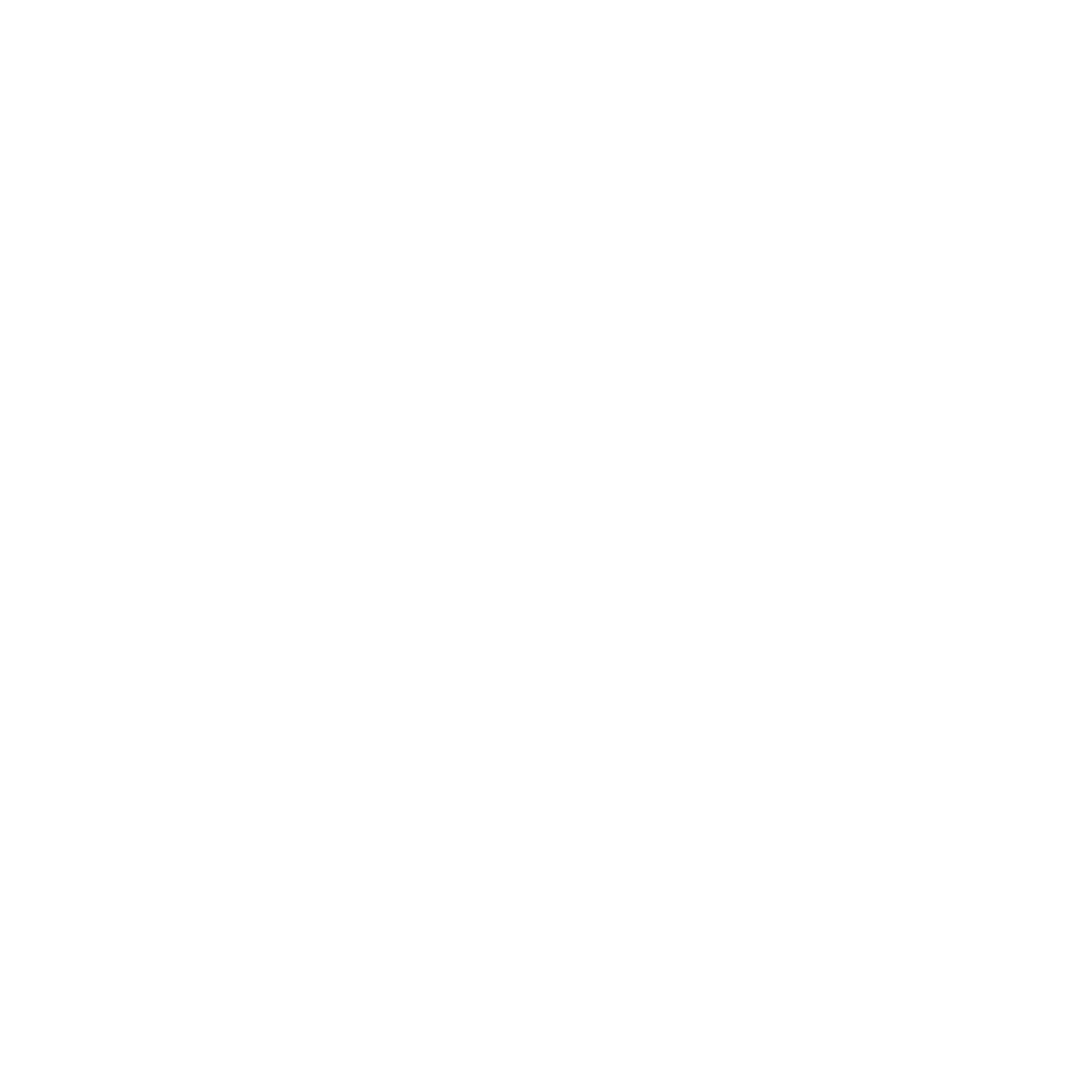
Heat transfer in packed beds
In packed beds, heat is transferred by particle contact, by the gas in the gaps and by radiation across free lines of sight. The effectiveness of heat transfer depends on a number of parameters, such as the conductivity and surface properties of the particles in the case of heat conduction and the emission and absorption characteristics in the case of heat radiation. A body that reflects well in the infrared range heats up more slowly than a body that absorbs most of the radiation.
A two-dimensional model for bulk material particles in a packed bed that do not have contact with each other can be realized as shown in Figure 2 by an arrangement of rods, where the center rod is heated and then emits infrared radiation into its immediate surroundings accordingly.
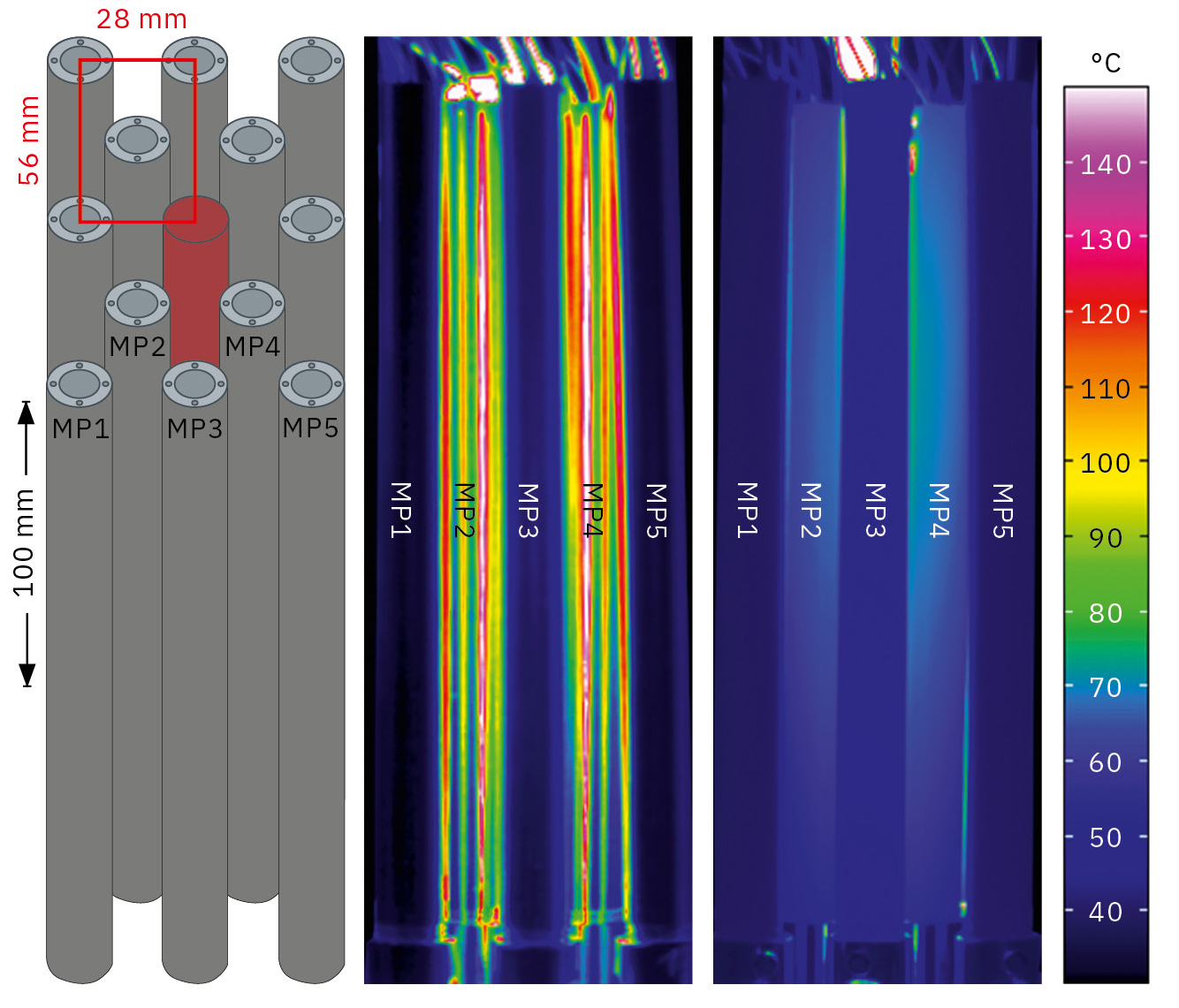
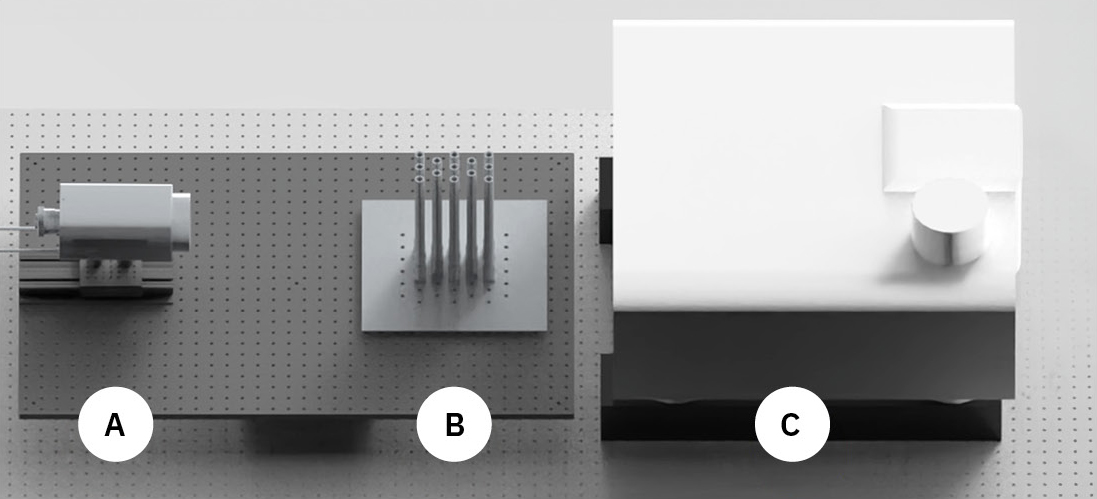
A heatable rod (MP0) is surrounded by 12 unheated rods made of the same material. The aim is to heat the heatable rod to 600 °C and use temperature and FTIR measurements to determine how quickly and how much the surrounding rods are heated indirectly through the radiated heat. This process was simulated using the discrete ordinates method (DOM) and Monte Carlo ray tracing (MCRT).[1]
Experiment setup
The imaging measurement of thermal radiation is possible with infrared cameras, and the emission spectrum can be investigated using FTIR spectroscopy. Tyslik et al. designed the unique experimental setup shown in Figure 3, which is presented here in more detail, to verify the heat transfer simulations with real data.
The key element is the arrangement of rods (B) described above. The temperature of the indirectly heated rods is investigated using a thermal imaging camera (A). The infrared radiation emitted is coupled into a modified Shimadzu IRTracer-100, replacing the factory-fitted light source. The radiation passes through the Michelson interferometer and the empty sample chamber and is measured using an MCT detector.
To define the field of view for this measurement and to focus the emitted light on the position of the original light source, two parabolic mirrors were used, as shown in Figure 4.
The lamp compartment of the spectrometer is indicated by the red border in Figure 4. A window made of potassium bromide (W1) closes off the spectrometer to the outside to protect it against humidity. A parabolic mirror (M1) collects the light emitted by one of the rods from Figure 2. Flat mirrors M2, M3 and M5 guide the light beam to the Michelson interferometer. Another parabolic mirror (M4) focuses on the position of the originally installed light source (G1) and takes images of the observed light spot on the factory-installed coupling mirror (CM1). The positioning of M1 and M2 determines the respective measuring point, i.e. the metal rod observed in each case.
Results
The measurement results are described in detail in references [1] and [2]. Stainless steel and magnesium oxide were used as the materials for the rods in this experimental investigation. A number of test parameters, such as the emissivity and the time required to reach the equilibrium temperature, were determined and discussed in detail. The central, directly heated rod radiates heat to the surrounding rods in the form of infrared radiation. This means these rods are heated indirectly and in turn emit heat in the form of infrared radiation but also reflect some of the infrared radiation.
With both materials and a target temperature of 600 °C for the heated rod, the temperature of all surrounding rods in this experiment stabilizes after approx. 50 minutes. As expected, the rods positioned closer to the center heat up faster and reach a much higher temperature. With stainless steel (Figure 2 center), the final temperature of the inner rods MP2 and MP4 reaches approx. 67 °C and that of the outer rods MP1 and MP5 is approx. 32 °C. Rod MP3 in the center heats up to 45 °C.
If the central rod is heated to a lower temperature, the emission intensity and the final temperature of the surrounding rods also decrease. As an example, the final temperatures of stainless steel rod MP2 at different heating temperatures are shown in Table 1 and the corresponding emission spectra in Figure 5.
Nr. |
MP0 [°C] |
MP2 [°C] |
T1 |
300 |
35 |
T2 |
400 |
46 |
T3 |
500 |
70 |
T4 |
600 |
73 |
The emission spectra of MP2 and MP3 after 60 minutes at a target temperature of 600 °C are shown in comparison for both of the test materials in Figure 6. MP2 has a temperature of 68 °C (steel) and 56 °C (MgO). MP3 has a temperature of 45 °C (steel) and 40 °C (MgO).
As expected, the emission intensity of the warmer rod MP2 is significantly greater than that of the colder rod MP3. From 1,000 to 4,500 cm–1, it is also significantly greater for stainless steel than for magnesium oxide. The emission spectrum of the stainless steel rod also shows a band from 1,600 to 2,500 cm–1, which was not observed in this form for either of the magnesium oxide rods or MP3.
However, the measurement results were influenced by reflections from the rod surfaces, particularly in the case of rod MP2, which was positioned far inwards.
To investigate the effects of reflection, the emission spectrum of the heatable rod was measured at 67 °C in a setup without the surrounding rods. In this experiment, the band observed in Figure 6 is not visible, as shown in Figure 7. This demonstrates just how much of the measured emission spectrum is caused by thermal radiation reflected from the surface. The results in [1] show that MCRT can reproduce the measurement results more accurately in simulations. However, the authors assume that in simulations of large real bulk materials, adaptation of the DOM is preferable as a computationally efficient compromise with adequate accuracy.[3] This approach will be validated against measurement results of the described setup.
Thermal conduction between the rods is prevented as far as technically possible in the setup described here, as the rods do not touch each other. In order to eliminate heat transfer by convection, a vacuum chamber will be added to the design in future.
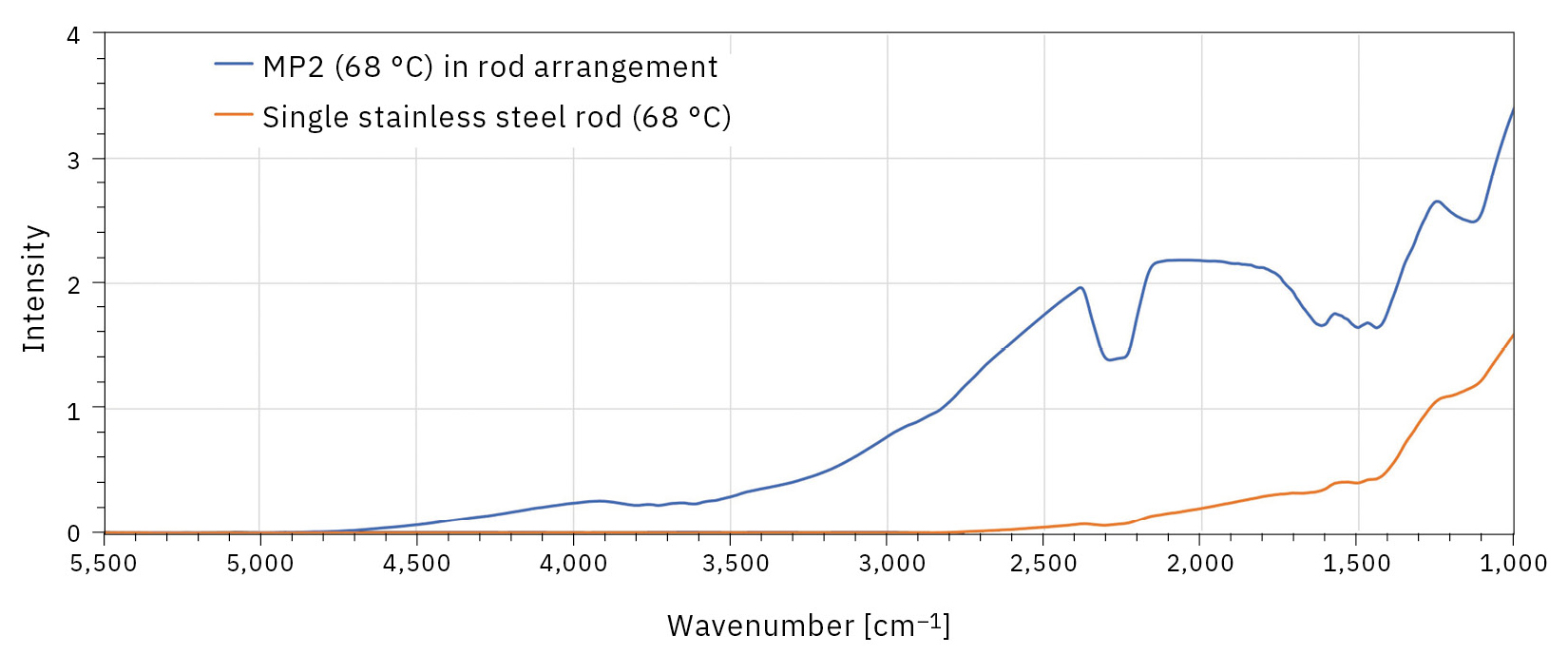
It all comes down to reflection
The described experiment setup with an arrangement of metal rods and a modified FTIR spectrometer allows heat transport by infrared radiation to be investigated. In a first experiment, stainless steel and magnesium oxide were compared, and the relevance of the reflective properties of the material was demonstrated. Due to the significantly higher reflectivity of the steel rods, the heat radiation penetrates a collection of steel rods easier than a collection of magnesium oxide rods. These initial results and the comparison with computer simulations provide important incentives for further research and modeling of heat transfer in packed beds. Precise knowledge of the mechanisms of this heat transfer helps optimizing a wide range of process parameters in large-scale factories and, as a result, save a great deal of energy. Areas of application could be lime burning or iron processing.
[1] Tyslik, M., Ebert, M., Schiemann, M., Wirtz, S., Lessig C. (2024). A numerical and experimental comparison of heat transfer in a quasi two-dimensional packed bed. Particuology. 84: 136–144. ISSN 1674-2001. https://doi.org/10.1016/j.partic.2023.03.014.
[2] Tyslik, M., Pörtner, L., Wirtz, S., Schiemann, M. (2024). Experimental investigation of radiative heat propagation in a simplified generic packed bed. Particuology. 88: 149–160. ISSN 1674-2001. https://doi.org/10.1016/j.partic.2023.09.005.
[3] Jaeger, B., Tyslik, M., Wirtz, S., Schiemann, M. (2022). Investigation of the radiative heating of cubic particles with DEM/CFD and the BO/DO approach. Powder Technology. 403: 117424. https://doi.org/10.1016/j.powtec.2022.117424.